The visual strategy specific to humans among hominids: A study using the gap-overlap paradigm
Fumihiro Kano, Satoshi Hirata, Josep Call, Masaki Tomonaga
DOI: 10.1016/j.visres.2011.09.006Abstract
Although an extensive body of literature exists on the cognitive underpinnings of gaze movements in macaques and humans, few studies have investigated this topic from a broader evolutionary perspective. This study used the gap-overlap paradigm to examine the timing of the gaze movements by four hominid species: humans, chimpanzees, gorillas, and orangutans. The saccade latency involved in shifting the gaze from central to peripheral stimuli was measured and compared under two conditions, gap and overlap. The central stimulus disappeared shortly before the onset of the peripheral stimulus under the gap condition, but it remained under the overlap condition. Although all species demonstrated similar saccade latencies under the gap condition, the species clearly differed from one another under the overlap condition, which may suggest their similar perceptual and motor mechanism of making a saccade on the one hand and their differential strategies for coping with the competition between two activities involving fixation and initiation of a saccade (i.e. central vs. peripheral visual stimuli) on the other hand. In particular, humans showed longer saccade latency under the overlap condition compared to the other great apes, which may reflect this species unique means of visual processing.
1. Introduction
Eye-gaze movement constitutes one of the most comprehensively studied visually guided behaviors displayed by humans and macaque monkeys. The visual strategy common to human and nonhuman primates involves the alternation of fixation and saccade; fixation involves maintaining certain parts of the visual field fixed on the fovea, which optimizes retinal acuity and color sensitivity, whereas saccades involve bringing new parts of the visual field onto the fovea using rapid eye movements. Given that primates retrieve visual information primarily from the fovea, how they move their gaze inform us about the ways in which visual information from the external world is retrieved and processed, an operation that is critically important to survival.
When primates shift their gaze from one location to another via saccadic eye movements, competition occurs between two mutually exclusive activities: fixation and saccade initiation. Resolving this competition consumes time because it involves various perceptual and cognitive processes (Findlay & Walker, 1999). This time-consuming competitive process can be examined using a simplified experimental arrangement known as the gap–overlap paradigm. An extensive body of literature exists with regard to the use of this paradigm in humans ( [Braun and Breitmeyer, 1988], [Kalesnykas and Hallett, 1987] and [Saslow, 1967]) and macaque monkeys ( [Baizer and Bender, 1989], [Fischer and Boch, 1983] and [Fischer and Weber, 1993]). Following this paradigm, a central (fixated) and a peripheral target stimulus appear sequentially on a computer screen under two conditions. The central fixation stimulus disappears after a short period of time (200–400 ms) before the target is presented under the gap condition, whereas the central fixation stimulus remains under the overlap condition. The time between target presentation and initiation of a saccade directed at the target is then measured (i.e., the saccade latency). In humans and monkeys, the saccade latency in response to peripheral stimuli has tended to be longer under the overlap than under the gap condition (known as the “gap effect”).
One well-established model of saccade generation (Findlay & Walker, 1999) assumes that resolution of the competition between fixation and saccadic activities requires the integration of various competing information signals to decide whether and where a saccade should occur. This model suggests that resolving this competition involves a relatively slow buildup in one activity and a decline in the other. Thus, when the saccadic activity overcomes the fixation activity, a saccade is generated. The reduction of fixation activity is termed disengagement. Physiologically, this competitive interaction can be observed in a subcortical area, the superior colliculus, where a decline in fixation neurons and a buildup in saccade-related neurons occur ( [Dorris and Munoz, 1995], [Dorris et al., 1997], [Munoz and Wurtz, 1993a] and [Munoz and Wurtz, 1993b]). Saccade generation is also controlled by various cortical areas including the parietal and frontal cortex ( [Munoz and Everling, 2004] and [Muri et al., 1998]), especially the frontal eye field ( [Dias and Bruce, 1994] and [Hanes and Schall, 1996]). According to Findlay and Walker’s (1999) model, the gap effect occurs because the fixation activity is automatically reduced by the offset of the fixation stimulus under the gap but not the overlap condition. The offset of the fixation stimulus under the gap condition also works as a warning signal that provides temporal information about the appearance of the target ( [Ross and Ross, 1980] and [Ross and Ross, 1981]).
It is well known that human infants from 1 to 4 months of age have difficulty in shifting their gaze to peripherally presented stimuli, the so called “obligatory fixation” (Stechler & Latz, 1966). The gap–overlap paradigm has revealed that infants in their first year of life show an earlier maturation of saccade latency under the gap than under the overlap condition (i.e., a larger gap effect in younger infants) ( [Farroni et al., 1999], [Hood and Atkinson, 1993] and [Matsuzawa and Shimojo, 1997]). Thus, it is suggested that human infants have difficulty in disengaging attention or reducing fixation activity under the overlap condition, in which such disengagement does not occur in an automatic manner. Physiologically, this phenomenon can be explained by the earlier maturation of subcortical compared with cortical regulatory systems (e.g., the frontal eye field; (Johnson, 1990). Similar difficulties with disengagement under the gap–overlap paradigm have also been reported among individuals with autism (Landry & Bryson, 2004).
Numerous studies have been conducted in macaque monkeys to examine the neural and behavioral mechanisms underlying saccade generation. Lesion studies, behavioral testing, functional neuroimaging studies, single-unit recordings, and anatomical studies in macaques and humans have shown that the neural circuitry controlling saccadic eye movements is homologous, or qualitatively similar, in the two species (Munoz & Everling, 2004). Studies comparing human and nonhuman primates have reported that trained macaques demonstrated a shorter saccadic latency than did trained humans (Baizer & Bender, 1989). During free viewing of a naturalistic dynamic scene, macaques scanned the scene more rapidly than did humans by shifting their gaze to the next location at an earlier time ( [Berg et al., 2009] and [Shepherd et al., 2010]).
A similar species difference was observed in comparisons of humans with one of their closest living primate relatives, chimpanzees (Pan troglodytes), when freely viewing static scenes (Kano & Tomonaga, 2009). A subsequent study (Kano & Tomonaga, in press) confirmed that this species difference in the timing of gaze movements did not depend on the nature of the stimuli (a scene containing humans/chimpanzees, fruit trees, only background, or texture) and thus seemingly reflected general patterns of gaze movements rather than specific responses to particular components of scenes. That subsequent study also examined the pattern of gaze movements in chimpanzees and humans using the gap–overlap paradigm under free-viewing conditions (no instruction/training) and found that chimpanzees and humans showed very similar saccadic latencies under the gap condition, but that chimpanzees shifted their gaze to the peripheral target at an earlier time than did humans under the overlap condition (i.e., a smaller gap effect in chimpanzees). The species similarity under the gap condition suggested that perceptual and motor abilities for making a saccade were comparable in both species, and the species differences under the overlap condition suggested the operation of differential visual strategies for resolving the competition between fixation and initiation of a saccade. In this context, it might be argued that humans follow a different pattern than do other primates in the timing of their gaze movements and that this species difference may derive from humans’ specific visual strategy for dealing with the aforementioned competition.
Despite their value, the current data have several shortcomings. First, there is the issue of the representativeness of the existing samples. Kano and Tomonaga (in press) compared six chimpanzees with 18 humans. However, the inclusion of additional individuals would be necessary to confirm that these results reflect species rather than individual differences. This is particularly important considering that these particular chimpanzees were previously extensively trained in computerized tasks, some of which required rapid responses to stimuli presented on a screen. Although these subjects were never trained to make saccades, confirmation of the validity of the free-viewing paradigm as a way to reveal spontaneous viewing patterns would require replication of the aforementioned results with chimpanzees with different training experiences.
Second, there is the issue of the type of stimuli presented in the tasks. Using the gap–overlap paradigm, Kano and Tomonaga (in press) presented naturalistic figures, faces, and objects rather than simple geometric figures to attract the apes’ and humans’ spontaneous attention to the stimuli. They found a minimal effect of different types of stimuli on species differences in saccade latencies, even though both species discriminated faces from objects in their gaze responses (in an experimental situation facilitating competition between the two stimulus types). Thus, it was suggested that species differences reflected general (or habitual) patterns of saccade generation rather than the immediate outcomes of the processing of meaningful stimuli. However, one might argue that faces and objects are both meaningful and that another type of stimulus, such as a meaningless figure, would be necessary to confirm these findings.
Finally, the investigation of species of great apes other than chimpanzees can contribute to clarifying the evolution of gaze-scanning patterns. As indicated above, the ways in which primates move their gaze can inform us about how they retrieve and process visual information that may be critically important for their survival. Thus, it is expected that each species’ gaze-scanning pattern sensitively reflects the phylogenetical and socioecological constraints specific to each species. Phylogenetically, chimpanzees are the closest to humans, followed by gorillas and orangutans. Thus, the comparison with the other apes may clarify whether the differences between chimpanzees and humans represent derived or ancestral traits. Additionally, each species has a differential socioecological background (Clutton-Brock & Harvey, 1977). Thus, our comparative study may also help us to assess the potential impact of socio-ecological variables on the gaze-scanning patterns.
The aim of the current study was to examine the timing of gaze movements from comparative perspective using the gap–overlap paradigm. We tested humans and three nonhuman great ape species, chimpanzees, gorillas and orangutans, living in three different facilities. Additionally, we investigated the effect of the type of stimulus. Following previous studies, we presented faces and objects, but also included a meaningless figure (texture).
2. Method
2.1. Participants
Four female gorillas (one adult, one infant, and two juveniles) and seven orangutans (one adult male, one infant male, four adult females, one juvenile female) housed at the Wolfgang Köhler Primate Research Center (WKPRC) at the Leipzig Zoo in Germany, eight chimpanzees (two adult males, three adult females, three juvenile females) housed at the Great Ape Research Institute (GARI) at Hayashibara Biomedical Laboratories, Inc. in Japan, and eight chimpanzees (two adult males, six adult females) housed at the Primate Research Institute at Kyoto University (KUPRI) participated in this study. Additionally, 16, six, and 18 humans (all adults) were recruited from WKPRC (all Europeans; six males, 10 females), GARI (all Japanese; two males, four females), and KUPRI (all Japanese; six males, 12 females), respectively, to participate in this study. Thus, 27 apes and 40 humans participated in this study. The data from six of the eight chimpanzees and the 18 humans at KUPRI were previously published (Kano & Tomonaga, in press). Two additional chimpanzees (an adult male and an adult female) were tested at KUPRI to increase the number of participants. All apes lived in social groups in a large outdoor compound attached to an indoor residence with regular feedings, enrichment, and water ad libitum. All apes were neither food- nor water-deprived. All apes and humans voluntarily participated in the study. Animal husbandry and research at WKPRC complied with the “European Association of Zoos and Aquaria (EAZA) Minimum Standards for the Accommodation and Care of Animals in Zoos and Aquaria” and the “World Association of Zoos and Aquariums (WAZA) Ethical Guidelines for the Conduct of Research on Animals by Zoos and Aquariums,” respectively. Animal husbandry at GARI and KUPRI complied with the “Care and Use of Laboratory Animals of Hayashibara Biochemical Laboratories, Inc.” and the 2002 version of the “Guidelines for the Care and Use of Laboratory Primates of the Primate Research Institute, Kyoto University,” respectively. Research conducted at GARI and KUPRI was approved by the Animal Welfare and Care Committee of KUPRI and the Animal Research Committee of Kyoto University. Informed consent was obtained from all human participants.
Chimpanzees housed at KUPRI had extensive experience with participation in computerized tasks using a touch-panel display, typically 15–21 in. in size, that required them to respond by touching geometrical or naturalistic figures appearing on the screen (Matsuzawa, Tomonaga, & Tanaka, 2006). Chimpanzees at GARI also had experience participating in such touch-panel experiments, but to a lesser extent than those at KUPRI (Idani & Hirata, 2006). Gorillas housed at WKPRC had begun participation in touch-panel experiments only recently, and orangutans at this facility had neither experienced such experiments nor been exposed to images on a computer screen. None of the apes or humans had been explicitly trained to shift their gaze rapidly.
2.2. Apparatus
The same eye-tracking techniques were used for apes and humans to ensure the same eye-tracking accuracy among species (Fig. 1a–c). However, we slightly modified the experimental arrangement in each facility to compensate for the specific constraints and capitalize on the particular resources already present at each institution. Eye-tracking experiments had been previously established with the chimpanzees at GARI and KUPRI ( [Hattori et al., 2010], [Hirata et al., 2010], [Kano and Tomonaga, 2009], [Kano and Tomonaga, 2010], [Kano and Tomonaga, 2011] and [Kano and Tomonaga, in press]) and had recently been introduced to apes at WKPRC. All apes were tested in an experimental booth. The eye-tracking apparatus and experimenter were located outside the booth and were separated from the apes via transparent acrylic panels at WKPRC and KUPRI. At GARI, the apparatus and experimenter, who was highly familiar to the apes, stayed inside the booth. An eye tracker with an infrared corneal reflection system measured participants’ gaze movements. We used a table-mounted apparatus at WKPRC and KUPRI (60 Hz; Tobii X120, Tobii Technology AB, Stockholm, Sweden) and a monitor-integrated type at GARI (60 Hz; Tobii T60), both of which were based on the same technology and thus possessed the same eye-tracking performance. These eye trackers were equipped with wide-angle lenses (±40° in the semicircle above the eye camera) and recorded both eyes, thereby allowing relatively large head movements by participants. The eye tracker and 17-in. LCD monitor (1280 × 1024 pixels at WKPRC and KUPRI and 1024 × 768 pixels at GARI) were mounted on a movable platform, and the distance between the platform and the participants was adjusted to the point at which gaze was most accurately recorded (approximately 60 cm). This flexible adjustment of the distance between the platform and the participants enabled us to record the gaze movements of apes without any head-restraining device. Four of the 11 apes (all juveniles) at WKPRC had difficulty approaching the panel upon the request of experimenter. For this reason, we used a nozzle and a tube attached to the panel, which continuously produced drops of grape juice during the experiment, thereby keeping the participants’ heads in front of the panel. Although they were sipping grape juice during the presentation of stimuli, they did not attend to the nozzle but freely moved their eyes. The experimenter at GARI sat beside the apes and held their heads lightly during the recordings. The other apes at WKPRC and KUPRI sat still in front of acrylic panels, and the experimenter encouraged them to face the eye tracker. The apes received small pieces of fruit ad libitum before and after the calibration procedure and presentation of pictures. No reward was given to reinforce any particular gaze behavior. At KUPRI, humans were tested in the same experimental booth as apes, whereas humans were tested in another room at GARI and WKPRC. Although the eye tracker recorded the eyes of humans and apes at KUPRI and those of the apes at WKPRC through the transparent acrylic panel, we confirmed that the acrylic panels (1.5–2 cm thick, absent of dirt or scratches) had no influence on the eye-tracking data in the preliminary test for accuracy. Each participant’s gaze was recorded as a relative coordinate with respect to the monitor size (i.e., not as the gaze angle). One degree of gaze angle corresponded to approximately 1 cm on the screen at a typical 60-cm viewing distance.
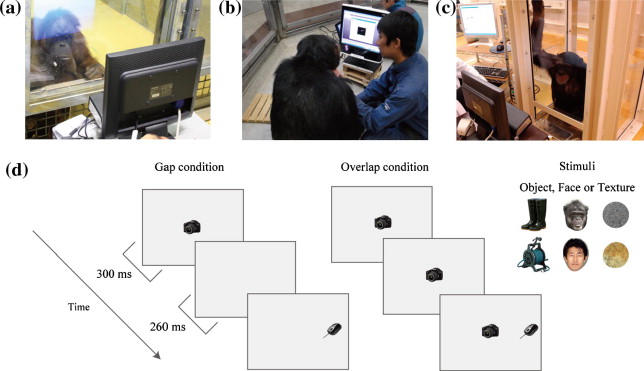
Fig. 1. (a-c) An ape on an eye tracker at WKPRC (Wolfgang Kohler Primate Research Center, Germany), GARI (Great Ape Research Institute, Japan), and KUPRI (Primate Research Institute, Kyoto University, Japan), respectively. (d) Gap?overlap paradigm used in this study.
An automated sequential calibration procedure was conducted for both apes and humans. Five-point calibration was used for humans, but the calibration points were reduced to two for apes to avoid interruption of the automated calibration process by participants averting their gaze elsewhere. We asked humans to fixate on the small dot appearing on each calibration point. For apes, we presented a small object or image at the calibration point for apes (a piece of fruit or a small video clip; approx. 1–2° in width/height), thereby drawing their spontaneous attention to the point. The calibration was repeated for the apes until maximum accuracy was obtained. The calibration accuracy was checked post hoc by presenting a small object or image at several points on the screen and manually monitoring the participants’ gaze toward those points. To reduce the time required for a daily session, the same calibration data were used for apes on separate days when the same level accuracy was achieved at the beginning of a daily session. To avoid any calibration error due to changes in posture or eye surface, the calibration accuracy was checked several times during the daily session, and the calibration was repeated when the same accuracy was not obtained. To quantitatively estimate the positional error, we conducted a preliminary session for each ape and human, in which we recorded the position of the participant’s gaze on the small object or image. We then calculated the distance between the center of object/image and the recorded gaze position. The error was found to be within 0.5–0.7°, on average, for all groups; this was sufficiently accurate for the requirements of this study. Daily sessions lasted for 10–15 min for each ape and human.
2.3. Procedure
Each trial began after participants focused on a small red mark appearing at a central position on the screen. We then presented a central fixation stimulus followed by a target stimulus (approx. 4.8 × 4.8° at a typical 60-cm viewing distance, approx. 9° apart). The target appeared randomly to the left or right 560 ms after the onset of the trial (Fig. 1d). We measured the time between target presentation and the initiation of a saccade directed at the target (i.e., the saccade latency). Under the gap condition, the central fixation stimulus disappeared 260 ms before target presentation, whereas the central fixation stimulus remained under the overlap condition. The peripheral target stimulus remained for 940 ms, and thus each trial lasted 1.5 s in total. Two types of stimulus, faces and objects, were initially used to test the GARI and KUPRI groups. Another stimulus type, texture, was also used to test the WKPRC group. Face stimuli included both ape and human faces. We prepared more than 50 exemplars of each stimulus type. Different exemplars of the same stimulus type were presented at both central and peripheral locations within each trial. Each exemplar was randomly selected from the entire pool of exemplars. A previous study conducted at KUPRI (Kano & Tomonaga, in press) involved six trials under each condition for each stimulus type (6 × 2 × 2 = 24 trials in total). Because that study confirmed the minimal variance across trials, we reduced the number of trials to three under each condition for each stimulus type at WKPRC (3 × 2 × 3 = 18 trials in total) and GARI (3 × 2 × 2 = 12 trials in total).
We randomized the presentation order of conditions and stimulus type for each participant. The entire session was conducted on a single day for humans at GARI (12 trials) and KUPRI (24 trials) and on two separate days for humans at WKPRC (nine trials each day). Six trials were conducted each day for apes at all facilities (3, 2, and 4 days in total at WKPRC, GARI, and KUPRI, respectively). Preliminary analysis, however, revealed no significant effect of day among those apes and humans tested on separate days.
After the completion of the whole session, we repeated trials in which participants prematurely shifted their gaze before the onset of the peripheral target. If the same occurred in those repeated trials, we excluded those trials from the analysis. This procedure resulted in the total data loss of 0.0%, 1.3% and 19.8% of all trials for humans, gorillas, and orangutans, respectively, at WKPRC; 6.9% and 11.4% for humans and chimpanzees, respectively, at GARI; and 0.2% and 2.0% for humans and chimpanzees, respectively, at KUPRI. We found no bias for a particular stimulus type or condition in those excluded trials. Additionally, for the quantitative analysis, we excluded the trials in which the saccade latency of participants was longer than the average for all trials (281 ms) plus 2.5 standard deviations (274 ms; i.e., longer than 555 ms) or in which the participants did not shift their gaze by the end of a trial. This resulted in the total data loss of 7.6%, 1.4%, and 0.0% of all trials for humans, gorillas and orangutans, respectively, at WKPRC; 4.4% and 4.7% for humans and chimpanzees, respectively, at GARI; and 4.8% and 1.5% for humans and chimpanzees, respectively, at KUPRI. These trials appeared primarily under the overlap condition, which probably reflects a characteristic of that condition, as discussed below. We found no bias for a particular stimulus type in these excluded trials.
3. Results
Fig. 2 presents the saccade latency of participants as a function of stimulus type (face, object, and texture) and condition (gap and overlap). We conducted three separate analyses of variance (ANOVAs) for the WKPRC, GARI, and KUPRI groups. We found a significant interaction between condition and species in the WKPRC group (gorillas, orangutans, and humans) (F(2, 24) = 11.10, P < 0.001, η2 = 0.48), which was explained by the difference in saccade latency among the species being more evident under the overlap (F(2, 24) = 23.15, P < 0.001, η2 = 0.65) than under the gap (F(2, 24) = 3.92, P = 0.033, η2 = 0.24) condition. Comparisons between orangutans and humans, between gorillas and humans, and between gorillas and orangutans showed interactions between condition and species that were significant (F(1, 21) = 19.50, P < 0.001, η2 = 0.48), not significant (F(1, 18) = 2.12, P = 0.16, η2 = 0.10), and marginally significant (F(1, 9) = 4.80, P = 0.056, η2 = 0.34), respectively. The analysis by species showed that the effect of condition was significant for humans (F(1, 15) = 159.97, P < 0.001, η2 = 0.91) and gorillas (F(1, 3) = 615.80, P < 0.001, η2 = 0.99) and marginally significant for orangutans (F(1, 6) = 4.54, P = 0.07, η2 = 0.43). The effect of stimulus type was not significant, either main effect or interaction with condition (P > 0.05).
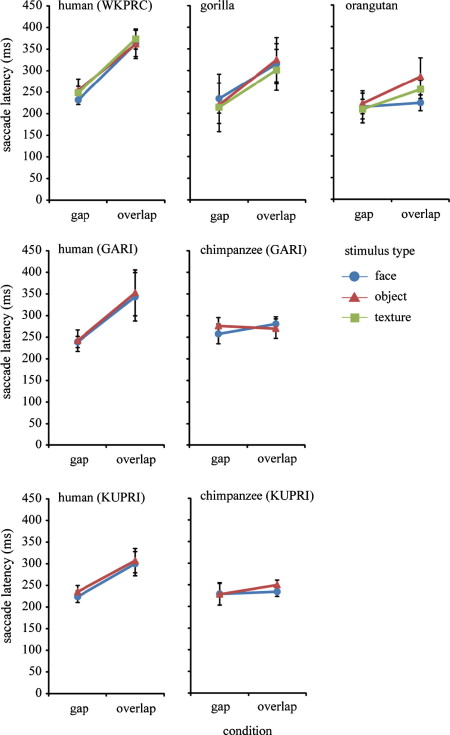
Fig. 2. Saccade latency (ms) as a function of condition (gap and overlap) and stimulus type (face, object, and texture) in humans, chimpanzees, gorillas, and orangutans from three research facilities. Error bars represent ±95% confidence intervals.
We found a significant interaction between condition and species at GARI (chimpanzees and humans) (F(1, 12) = 14.51, P = 0.002, η2 = 0.54), which can be explained by the species difference in saccade latency being more evident under the overlap (F(1, 12) = 7.72, P = 0.017, η2 = 0.39) than the gap (F(1, 12) = 3.77, P = 0.076, η2 = 0.23) condition. The analyses by species showed that the effect of condition was significant for humans (F(1, 5) = 24.17, P = 0.004, η2 = 0.82) but not for chimpanzees (F(1, 7) = 0.71, P = 0.42, η2 = 0.093). The effect of stimulus type was not significant, either main effect or interaction with condition (P > 0.05).
We found a significant interaction between condition and species at KUPRI (chimpanzees and humans) (F(1, 24) = 15.72, P = 0.001, η2 = 0.39), which can be explained by the species difference in saccade latency being more evident under the overlap (F(1, 24) = 8.38, P = 0.008, η2 = 0.25) than under the gap (F(1, 24) = 0.002, P = 0.96, η2 < 0.001) condition. The analyses by species showed that the effect of condition was significant for both humans (F(1, 17) = 59.05, P = 0.004, η2 = 0.77) and chimpanzees (F(1, 7) = 12.25, P = 0.010, η2 = 0.63), although the difference was small for chimpanzees (13 ms). We found a significant effect of stimulus type (F(1, 24) = 5.69, P = 0.025, η2 = 0.19) in the KUPRI group, although the difference was small (8 ms).
We did not have a sufficient number of individual samples to examine the effects of age and sex, but the exclusion of juveniles and males did not alter the aforementioned statistical results. The effect of laterality (either right or left) was not significant in terms of either main effects or interactions (P > 0.05) at any of the facilities. We confirmed the same statistical results even when we limited the analyses to the first six trials at all facilities.
Fig. 3 presents the distribution of saccade latencies from 0 to 500 ms. Apes and humans showed a skewed distribution in their saccade latencies, with the peaks around 200 ms. The distributions were similar across species under the gap condition. In contrast, the distribution was skewed more leftward in apes than in humans, and the distribution of the saccade latencies in humans was characterized by a long right tail exceeding 300 ms under the overlap condition. This species difference was most pronounced between humans and chimpanzees/orangutans, and the results for gorillas were between these two extremes. See Table 1 for the distribution of saccade latency of individuals.
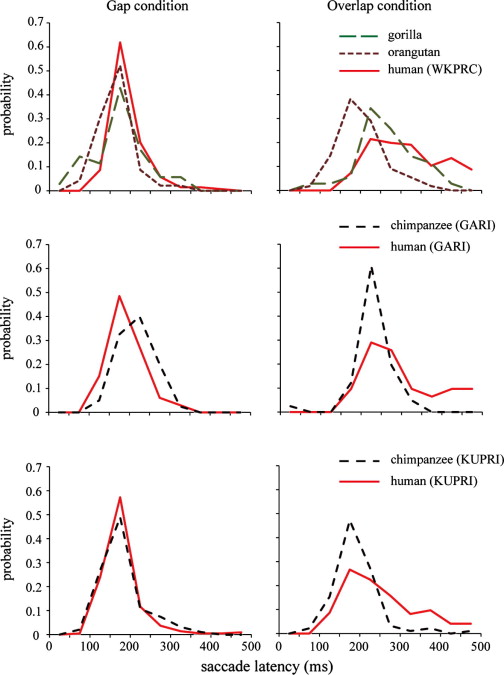
Fig. 3. Frequency distribution of saccade latencies from 0 to 500 ms in four species from three research facilities. The data were pooled for all participants and stimulus types. The bin was 50 ms.
151–200 | 201–250 | 251–300 | 301–350 | 351–400 | 401–450 | Total | |
---|---|---|---|---|---|---|---|
Gap condition | |||||||
WKPRC | |||||||
Human | 7 | 9 | 16 | ||||
Gorilla | 1 | 2 | 1 | 4 | |||
Orangutan | 2 | 5 | 7 | ||||
GARI | |||||||
Human | 4 | 2 | 6 | ||||
Chimpanzee | 2 | 4 | 2 | 8 | |||
KUPRI | |||||||
Human | 3 | 12 | 3 | 18 | |||
Chimpanzee | 1 | 5 | 1 | 1 | 8 | ||
Overlap condition | |||||||
WKPRC | |||||||
Human | 5 | 8 | 3 | 16 | |||
Gorilla | 2 | 1 | 1 | 4 | |||
Orangutan | 4 | 2 | 1 | 7 | |||
GARI | |||||||
Human | 2 | 2 | 2 | 6 | |||
Chimpanzee | 1 | 5 | 2 | 8 | |||
KUPRI | |||||||
Human | 4 | 4 | 6 | 2 | 2 | 18 | |
Chimpanzee | 6 | 1 | 1 | 8 |
4. Discussion
Humans, chimpanzees, gorillas, and orangutans from three research facilities were compared in terms of their saccade latencies using the gap–overlap paradigm. Although all species showed similar saccade latencies under the gap condition, the species clearly differed from one another under the overlap condition. In general, humans showed longer saccade latencies did than the other apes under that condition, which is explained by saccade latencies longer than 300 ms being more frequent in humans than in other apes. We found little evidence that stimulus type affected latencies.
The similarities among the various species under the gap condition suggest common perceptual and motor abilities for responding to peripherally presented stimuli (e.g., the sensitivity to salience of peripheral vision, the execution of saccadic eye movements). Relatively consistent saccade latencies under the gap condition have also been observed among human infants at various developmental stages ( [Hood and Atkinson, 1993] and [Matsuzawa and Shimojo, 1997]) and in autistic and typically developing children (Landry & Bryson, 2004). These phylogenetic, developmental, and clinical consistencies suggest a relatively primitive or fundamental operation underlying saccade generation under the gap condition.
In contrast, humans and apes differed under the overlap condition. Unlike chimpanzees and orangutans, humans showed a clear overlap (or gap) effect. Interestingly, gorillas showed a pattern that was somewhat similar to that displayed by humans in this regard. Given that the species were similar in their saccade latencies under the gap condition, the species differences under the overlap condition suggest the use of differential strategies for dealing with the competition between fixation and initiation of a saccade. An alternative account is that the participants anticipated the appearance of the target via the offset of the fixation stimulus (or the gap) ( [Reuter-Lorenz et al., 1991] and [Ross and Ross, 1980]). However, this account is unlikely in the context of this study because any of our participants were not trained in the task, as will be discussed in greater detail below.
Our study investigated participants’ spontaneous (or “natural”) pattern of gaze shifting rather than their ability to control their gaze. Thus, they viewed the stimuli freely without any instruction or training and without any head-restraining device. This arrangement differed critically from those used in previous studies with instructed/trained humans and macaques as subjects (e.g., [Fischer and Boch, 1983] and [Fischer and Ramsperger, 1984]) and resembles those used in previous studies with untrained human infants as subjects ( [Hood and Atkinson, 1993] and [Matsuzawa and Shimojo, 1997]). Several lines of evidence indicate that the participants in this study showed their spontaneous patterns of gaze shifting. First, differing amounts of experience in participating in computerized tasks or exposure to computer screens did not affect the data obtained from apes. Second, the different reward schedule for apes (receiving a reward for participating in the experiments, but not for their gaze behaviors) did not affect the results of this study. Third, we observed few express or anticipatory saccades (fewer than 100 ms), phenomena that have been frequently observed in trained subjects when the location at which the target appeared was predictable (Fischer & Weber, 1993). Finally, analysis of the first six trials of the session, in which an effect for (uninstructed) training or learning was unlikely, yielded results identical to those for the entire session. Interestingly, the untrained humans in this study showed a skewed distribution of saccade latency, with a long right tail extending beyond 300 ms under the overlap condition, which has been commonly observed in humans who were freely viewing naturalistic scenes. In contrast, trained humans in the previous studies have often shown a symmetrical or inverted bell-shaped distribution of saccade latency under the overlap condition ( [Braun and Breitmeyer, 1988], [Fischer and Weber, 1993] and [Reuter-Lorenz et al., 1991]).
Despite its theoretical importance, the free viewing design of this experiment may also have shortcomings given that the possible differences in the motivational states of each species may have had certain influence on the results (although we did not find any behavioral evidence to show such motivational differences). We also recognize that genuine natural patterns of gaze movements can be observed only during the course of daily activities. Thus, further studies simulating naturalistic contexts (e.g., use of head-mounted eye-tracking devices) (Land, Mennie, & Rusted, 1999) are necessary.
We found no effect of stimulus type on saccade latency. Thus, the saccade latency elicited by the gap–overlap paradigm in this study may have reflected a habitual or well-automated process for saccade generation rather than an immediate outcome of processing meaningful stimuli. Kano and Tomonaga (in press) found a similar result in chimpanzees and humans. That is, when an object and (a seemingly more salient) face were presented at central and peripheral locations, respectively, under one condition and in the opposite locations, respectively, under the other condition, the saccade latencies of both species were shorter when the object was presented centrally and the face was presented peripherally than vice versa, whereas the effect of overlap remained the same under both conditions. Therefore, although the saccade latency of participants seems to be influenced by the stimulus type, the effect of overlap seems to be influenced by the competition between the two stimuli, rather than by the stimulus type per se.
Somewhat surprisingly, among the nonhuman great apes, gorillas showed the clearest overlap effect. However, the small sample size (n = 4) precludes our reaching a definitive conclusion, and further studies are necessary to confirm this result. One interpretation for this possible species difference is the possible behavioral or cognitive uniqueness of gorillas among the great apes, which has been suggested by previous studies ( [Peignot and Anderson, 1999] and [Suarez and Gallup, 1981]). Alternatively, gorillas may have been somewhat neophobic to the presented stimuli. That is, their attention (or effective visual field) may have been temporarily narrowed to the central stimuli, rendering them less sensitive to the appearance of peripheral stimuli.
At least two ultimate (or evolutionary) interpretations are possible with regard to the benefits (and costs) of the adoption of such specific visual strategies by humans and apes. First, the specific visual strategy used by each species may have survival value in specific socioecological environments. For example, it may be more beneficial to scan visual fields more quickly by shifting gazes earlier in the context of arboreal living, where objects and animals tend to appear in an unexpected manner, as may be the case for chimpanzees and orangutans. To clarify the effect of socioecological factors, additional comparative studies in various primate species are necessary.
Second, the pronounced effect of overlap (or competition) in humans may reflect their unique means of information processing among hominids. That is, rather than constantly retrieving new information, humans may keep their gaze stationary and thereby promote time-consuming internal processing (e.g., for the sake of categorical and language processing). In contrast, apes may switch their focus of attention (i.e., the fovea) more frequently than humans and may thereby cover a wider visual field via gaze movements. Thus, a trade-off between the depth and breadth of information processing/retrieval may occur in human and nonhuman apes. However, two limitations must be considered with regard to this hypothesis. First, no quantitative information is available about the information retrieval/processing in these species in this study. Second, the hypothesis does not explain the effect of the overlap in gorillas (although the effect was somewhat smaller in gorillas than in humans). One could assume that this overlap effect in gorillas derived from a different cause than that in humans, as explained above; however, further studies are necessary to clarify this issue.
In conclusion, this study found phylogenetic similarities and differences in saccade latencies among hominid species. Although all species seem to have similar perceptual and motor mechanisms for performing saccades, the species may differ in their strategies for coping with the competition between two activities involving fixation and saccade initiation. In particular, humans seem to spend a longer time resolving this competition than the other great apes, which may reflect this species’ unique means of information processing.
Acknowledgments
This research was financially supported by the Japan Society for the Promotion of Science (JSPS) and the Ministry of Education, Culture, Sports, Science and Technology (MEXT) of Japan Grants-in-Aid for Scientific Research (Nos. 16002001, 19300091, 20002001, 20220004, 20680015, 212299, 23300103), the JSPS/MEXT global COE programs (D07 and A06), and the JSPS Institutional Program for Young Researcher Overseas Visits. We thank C. Rahn, F. Stock, H. Knofe, J. Corbit, R. Pieszek, and the other members of WKPRC as well as K. Fuwa, K. Sugama, K. Kusunoki, and S. Fujita for their help with the experiment. We also thank the Leipzig Zoo in Germany, the Hayashibara Biomedical Laboratory, Inc. in Japan, and the Center for Human Evolution Modeling Research at the Primate Research Institute in Japan for the daily care of the apes.
References
- J.S. Baizer, D.B. Bender Comparison of saccadic eye movements in humans and macaques to single-step and double-step target movements Vision Research, 29 (4) (1989), pp. 485–495
- D.J. Berg, S.E. Boehnke, R.A. Marino, D.P. Munoz, L. Itti Free viewing of dynamic stimuli by humans and monkeys Journal of Vision, 9 (5) (2009), pp. 1–15
- D. Braun, B.G. Breitmeyer Relationship between directed visual attention and saccadic reaction times Experimental Brain Research, 73 (3) (1988), pp. 546–552
- T.H. Clutton-Brock, P.H. Harvey Primate ecology and social organization Journal of Zoology, 183 (1) (1977), pp. 1–39
- E.C. Dias, C.J. Bruce Physiological correlate of fixation disengagement in the primate’s frontal eye field Journal of Neurophysiology, 72 (5) (1994), pp. 2532–2537
- M.C. Dorris, D.P. Munoz A neural correlate for the gap effect on saccadic reaction times in monkey Journal of Neurophysiology, 73 (6) (1995), pp. 2558–2562
- M.C. Dorris, M. Pare, D.P. Munoz Neuronal activity in monkey superior colliculus related to the initiation of saccadic eye movements Journal of Neuroscience, 17 (21) (1997), pp. 8566–8579
- T. Farroni, F. Simion, C. Umilt, B.D. Barba The gap effect in newborns Developmental Science, 2 (2) (1999), pp. 174–186
- J.M. Findlay, R. Walker A model of saccade generation based on parallel processing and competitive inhibition Behavioral and Brain Sciences, 22 (04) (1999), pp. 661–674
- B. Fischer, R. Boch Saccadic eye movements after extremely short reaction times in the monkey Brain Research, 260 (1) (1983), pp. 21–26
- B. Fischer, E. Ramsperger Human express saccades: Extremely short reaction times of goal directed eye movements Experimental Brain Research, 57 (1) (1984), pp. 191–195
- B. Fischer, H. Weber Express saccades and visual attention Behavioral and Brain Sciences, 16 (03) (1993), pp. 553–567
- D.P. Hanes, J.D. Schall Neural control of voluntary movement initiation Science, 274 (5286) (1996), pp. 427–430
- Y. Hattori, F. Kano, M. Tomonaga Differential sensitivity to conspecific and allospecific cues in chimpanzees and humans: A comparative eye-tracking study Biology Letters, 6 (5) (2010), pp. 610–613
- S. Hirata, K. Fuwa, K. Sugama, K. Kusunoki, S. Fujita Facial perception of conspecifics: Chimpanzees (Pan troglodytes) preferentially attend to proper orientation and open eyes Animal Cognition, 13 (5) (2010), pp. 679–688
- B.M. Hood, J. Atkinson Disengaging visual attention in the infant and adult Infant Behavior & Development, 16 (4) (1993), pp. 405–422
- G. Idani, S. Hirata Studies at the Great Ape Research Institute, Hayashibara D.A. Washburn (Ed.), Primate perspectives on behavior and cognition, American Psychological Association, Washington (2006), pp. 29–36
- M.H. Johnson Cortical maturation and the development of visual attention in early infancy Journal of Cognitive Neuroscience, 2 (2) (1990), pp. 81–95
- R.P. Kalesnykas, P.E. Hallett The differentiation of visually guided and anticipatory saccades in gap and overlap paradigms Experimental Brain Research, 68 (1) (1987), pp. 115–121
- Kano, F., & Tomonaga, M. (in press). Species difference in the timing of gaze movement between chimpanzees and humans. Animal Cognition.
- F. Kano, M. Tomonaga How chimpanzees look at pictures: A comparative eye-tracking study Proceedings of the Royal Society of London Series B, 276 (1664) (2009), pp. 1949–1955
- F. Kano, M. Tomonaga Face scanning in chimpanzees and humans: Continuity and discontinuity Animal Behaviour, 79 (1) (2010), pp. 227–235
- F. Kano, M. Tomonaga Perceptual mechanism underlying gaze guidance in chimpanzees and humans Animal Cognition, 14 (3) (2011), pp. 377–386
- M. Land, N. Mennie, J. Rusted The roles of vision and eye movements in the control of activities of daily living Perception, 28 (11) (1999), pp. 1311–1328
- R. Landry, S.E. Bryson Impaired disengagement of attention in young children with autism Journal of Child Psychology and Psychiatry and Allied Disciplines, 45 (6) (2004), pp. 1115–1122
- M. Matsuzawa, S. Shimojo Infants’ fast saccades in the gap paradigm and development of visual attention Infant Behavior & Development, 20 (4) (1997), pp. 449–455
- T. Matsuzawa, M. Tomonaga, M. Tanaka Cognitive development in chimpanzees Springer, Tokyo (2006)
- D.P. Munoz, S. Everling Look away: The anti-saccade task and the voluntary control of eye movement Nature Reviews Neuroscience, 5 (3) (2004), pp. 218–228
- D.P. Munoz, R.H. Wurtz Fixation cells in monkey superior colliculus. I. Characteristics of cell discharge Journal of Neurophysiology, 70 (2) (1993), pp. 559–575
- D.P. Munoz, R.H. Wurtz Fixation cells in monkey superior colliculus. II. Reversible activation and deactivation Journal of Neurophysiology, 70 (2) (1993), pp. 576–589
- R.M. Müri, S. Rivaud, B. Gaymard, C.J. Ploner, A.I. Vermersch, C.W. Hess et al. Role of the prefrontal cortex in the control of express saccades. A transcranial magnetic stimulation study Neuropsychologia, 37 (2) (1998), pp. 199–206
- P. Peignot, J.R. Anderson Use of experimenter-given manual and facial cues by gorillas (Gorilla gorilla) in an object-choice task Journal of Comparative Psychology, 113 (3) (1999), pp. 253–260
- P.A. Reuter-Lorenz, H.C. Hughes, R. Fendrich The reduction of saccadic latency by prior offset of the fixation point: An analysis of the gap effect Attention, Perception, & Psychophysics, 49 (2) (1991), pp. 167–175
- L.E. Ross, S.M. Ross Saccade latency and warning signals: Stimulus onset, offset, and change as warning events Attention, Perception, & Psychophysics, 27 (3) (1980), pp. 251–257
- S.M. Ross, L.E. Ross Saccade latency and warning signals: Effects of auditory and visual stimulus onset and offset Attention, Perception, & Psychophysics, 29 (5) (1981), pp. 429–437
- M.G. Saslow Effects of components of displacement-step stimuli upon latency for saccadic eye movement Journal of the Optical Society of America, 57 (8) (1967), pp. 1024–1029
- S.V. Shepherd, S.A. Steckenfinger, U. Hasson, A.A. Ghazanfar Human–monkey gaze correlations reveal convergent and divergent patterns of movie viewing Current Biology, 20 (7) (2010), pp. 649–656
- G. Stechler, E. Latz Some observations on attention and arousal in the human infant Journal of the American Academy of Child and Adolescent Psychiatry, 5 (1966), pp. 517–525
- S.D. Suarez, G.G. Gallup Self-recognition in chimpanzees and orangutans, but not gorillas Journal of Human Evolution, 10 (2) (1981), pp. 175–188
Keywords
Eye-tracking, Gap-overlap, Great ape, Saccade latency,